J. N. Cross1, A. Niemi2, N. Steiner3, and D. J. Pilcher1,4
1Pacific Marine Environmental Laboratory, NOAA, Seattle, WA, USA
2Freshwater Institute, Fisheries and Oceans Canada, Winnipeg, MB, Canada
3Institute of Ocean Sciences, Fisheries and Oceans Canada, Sidney, BC, Canada
4Cooperative Institute for Climate, Ocean, and Ecosystem Studies, University of Washington, Seattle, WA, USA
Highlights
- Recent work has shown that the Arctic Ocean is acidifying faster than the global ocean, but with high spatial variability.
- A growing body of research indicates that acidification in the Arctic Ocean could have implications for the Arctic ecosystem, including influences on algae, zooplankton, and fish.
- Cutting-edge tools like computational models are increasing our capacity to understand patterns, trends, and impacts of ocean acidification in the Arctic region.
The uptake of anthropogenic carbon dioxide (CO2) causes a cascade of chemical reactions that decreases ocean pH and carbonate ion concentrations, a process known as ocean acidification (OA). While OA is a global process, some of the fastest rates of ocean acidification around the world have been observed in the Arctic Ocean (e.g., Qi et al. 2017, 2020). These extremely rapid rates of acidification reflect the Arctic’s natural vulnerability to changes in pH, caused by cold temperatures, naturally higher baseline CO2 concentrations resulting from global circulation processes, seasonal processes that rapidly concentrate CO2 in some water masses, as well as unique land-sea interactions and hydrological mechanisms (circumpolar perspective broadly reviewed by AMAP 2018). Surface waters in some parts of the Arctic Ocean are already undersaturated with respect to some biologically important calcium carbonate minerals (e.g., aragonite and calcite) and most regions of the Arctic are likely to become corrosive (able to dissolve biologically important carbonate minerals) by the end of the century (AMAP 2018). These changes could have serious implications for the regional ecosystem, including detrimental impacts on local wildlife, cultural assets and practices, and subsistence resources.
Robust sampling programs that prioritize the collection of OA data (e.g., pH, partial pressure of CO2, dissolved inorganic carbon (DIC), and total alkalinity (TA)) are extremely difficult to implement in the Arctic. The coastal sub-Arctic seas exhibit a highly dynamic spatial and temporal variability as the underlying biogeochemistry is impacted by a range of land, ocean, and atmosphere processes. Accordingly, mature OA monitoring systems must be highly resolved in both space and time to provide adequate information for decision support. Given the expansive area, the remote geographic location, and harsh winters, traditional monitoring tools are also challenging to deploy consistently in the Arctic region, although some of these time series are starting to mature (e.g., Beaufort Gyre: Zhang et al. 2020; Canadian Archipelago: Beaupré-Laperrière et al. 2020; Eurasian Basin: Ulfsbo et al. 2018; Fram Strait: Chierici et al. 2019; Svalbard: Jones et al. 2021).
Despite these advances, we do not have a synoptic understanding of OA across the pan-Arctic system. Accordingly, computational models grounded in observable data have emerged as a useful tool to help explore spatial-temporal variability due to their much finer spatial and temporal resolution. Using these outputs, researchers are better able to explore the intensity, duration, and extent of ecosystem exposure to OA processes. In recent years, regional and global modeling studies have been used to explore both long- and short-term aspects of OA in the Arctic (e.g., Bering Sea: Pilcher et al. 2019; pan-Arctic, Terhaar et al. 2020), as well as the processes leading to these trends that are notoriously difficult to observe (e.g., pan-Arctic sea-ice related impacts: Mortenson et al. 2020). However, there is substantial regional and seasonal variability especially where land processes can influence OA, highlighting potential problems with interpolating sparse measurements (e.g., Chierici et al. 2019; Jones et al. 2021). Better regional to local climate projections may provide key improvements. Model studies continue to be refined and will likely form a pivotal part of future Arctic OA research.
As the observational record of OA in the Arctic continues to grow, research on the possible impact of OA on Arctic ecosystems continues to progress both in the laboratory and in the field (Fig. 1). The primary concern is that the short food web linkages so characteristic of the Arctic may lead to widespread impacts of OA across the ecosystem, creating both winners and losers. This is evident at the very base of the food chain: for example, OA negatively affects the calcification of some Arctic phytoplankton (pan-Arctic: Ardyna and Arrigo 2020) and may shift the community toward smaller species (western Arctic: Sugie et al. 2020). Some primary producers may experience little impact; research syntheses indicate that OA likely has a limited effect on sea ice algae, given that the biogeochemistry of the ice matrix itself naturally undergoes extreme fluctuations that result in evolutionary resilience (central Arctic: Torstensson et al. 2021).
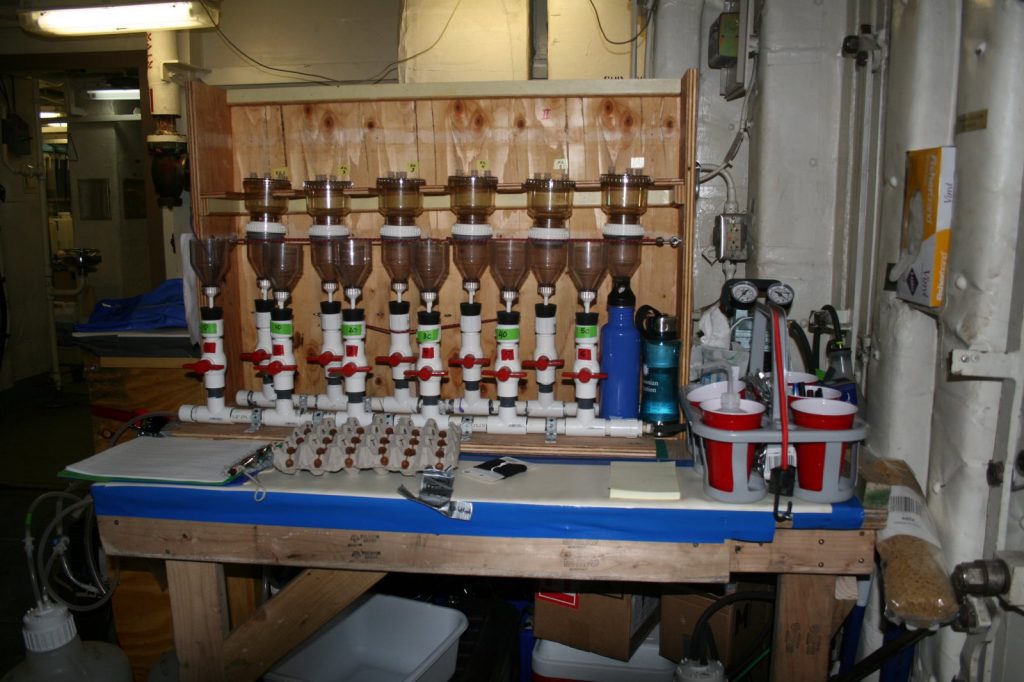
At the zooplankton trophic level, the quintessential species for detrimental OA impacts is the pteropod (sea snail). These organisms are extremely sensitive to ocean pH and are often used around the world as indicators that can inform OA conditions. Both laboratory and field observations have shown that pteropod responses to OA include reduced juvenile survival, reduced shell growth and condition, as well as costly metabolic regulation. Arctic population connectivity and morphological characteristics of pteropods is a growing area of research. For example, recent studies of natural populations indicate a high occurrence of severe shell dissolution in the Bering Sea, Amundsen Gulf, and Svalbard margin (Niemi et al. 2021; Bednaršek et al. 2021; Anglada-Ortiz et al. 2021, respectively). While pteropods are an important biological indicator, research on other organisms specific to Arctic ecosystems will also support regional relevance. For example, fish show sensitivities to OA, including important species such as Arctic cod (e.g., western Arctic cod populations: Steiner et al. 2019; eastern Arctic cod: Hänsel et al. 2020). However, key questions remain to fully understand the mechanisms that produce individual and population-level responses to OA. Across species (fish, benthic and pelagic invertebrates) repair, adaptability, and associated tolerance have been linked to resource availability (e.g., Niemi et al. 2021; Hänsel et al. 2020; Duarte et al. 2020; Goethel et al. 2017), indicating the importance of a holistic ecosystem approach to understand OA biological responses (Fig. 2).
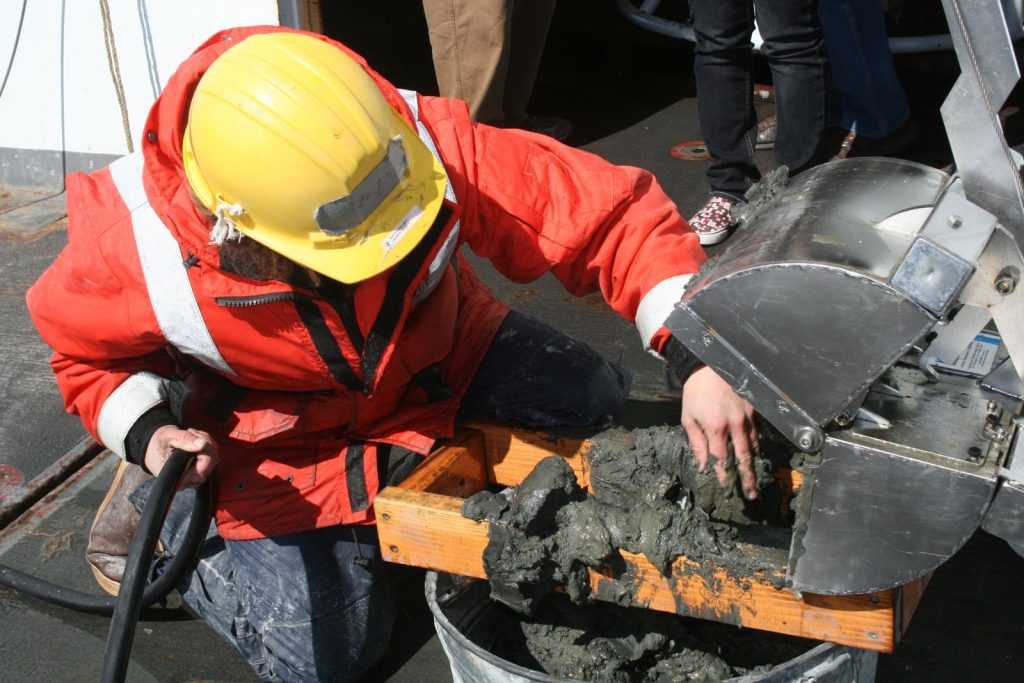
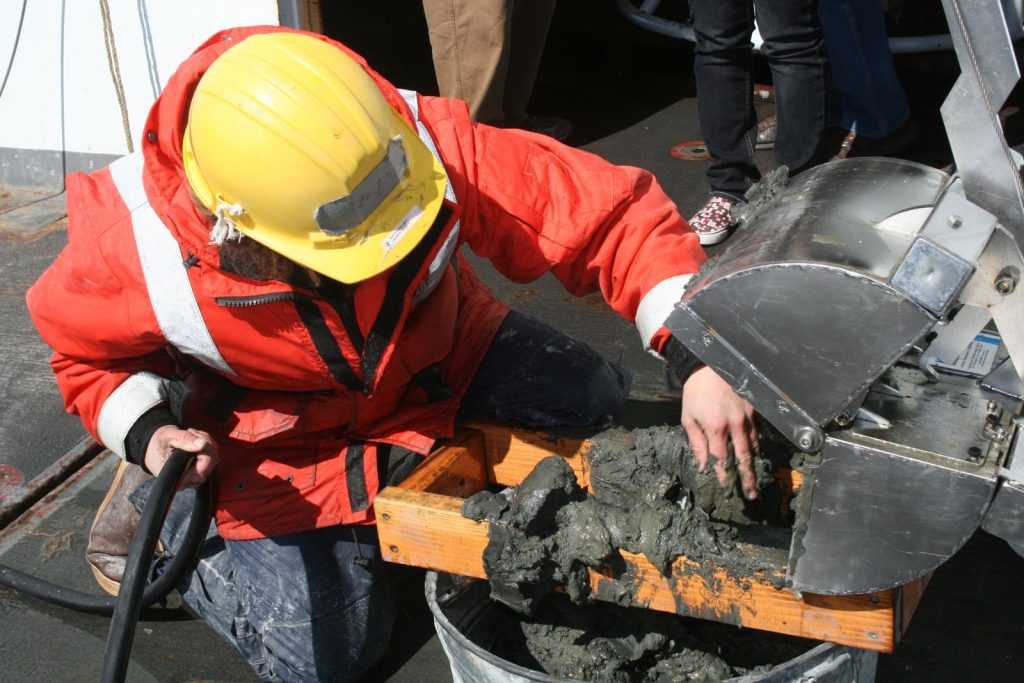
Seals, walrus, and marine birds may be impacted by the inherent vulnerability of their preferred foods to acidification. Calcifying bivalves are particularly sensitive to acidified conditions; weakly acidified waters can reduce growth, while severely corrosive waters can eventually begin to dissolve shells. Although laboratory experiments have identified resilience to acidification in Arctic invertebrates (Goethel et al. 2017), some model research suggests that they are likely to be among the most negatively impacted invertebrate populations in the world (Tai et al. 2021). Though the specifics remain uncertain, it is likely that the consequences of continuing OA will be detrimental for parts of the marine food web over the next decade. Warming and acidification are likely to become compounding stressors for the Arctic ecosystem by the end of the century. More research will be necessary to determine how acidification-stressed marine invertebrate populations may influence the Arctic ecosystem. This work will be especially important given that invertebrates as well as their predators are important commercial, cultural, and subsistence resources across the region.
Building ecosystem and human resilience to OA in the Arctic in part will require global solutions, given that OA is primarily caused by global carbon dioxide emissions. However, regional decision makers are likely to benefit from continually improving resolution of both data collection and regional modeling, which will provide additional support for Arctic decision makers and ecosystem management. Measurements from novel sensors, especially those collecting surface data are likely to improve the resolution of regional CO2 flux products and provide a better understanding of the surface CO2 sink for carbon across the Arctic. Additionally, short-term forecasting applications are likely to be developed for regional and pan-Arctic models in support of ecosystem management systems. Through this process, development of connections between biogeochemical and ecosystem models, based on empirical laboratory and in-situ data, are likely to allow researchers to continue to explore the impact of OA on regional ecosystems. The ultimate goal is to develop an interdisciplinary, hybridized approach that will allow the scientific community to develop annual OA products of the type now produced for temperature or sea ice extent for the Arctic Report Card. Given the high connectivity of processes leading to OA and its downstream effects, trans-national data access and collaboration will be essential for success.
In addition to improvements in data collection, it also seems likely that coastal marine CO2 removal may scale inside coastal waters in the Arctic. The IPCC has acknowledged that achieving climate goals requires substantial changes to atmospheric greenhouse gas concentrations. While emissions-reduction approaches are an essential component of addressing this challenge, negative emissions strategies will be necessary for keeping global temperatures at recommended levels (IPCC 2021). Negative emissions strategies refer to a portfolio of techniques that are used to manually remove greenhouse gases from the atmosphere and store them away from the atmosphere. Carbon dioxide removal (CDR) specifically references techniques that remove legacy emissions of CO2 from the atmosphere. Where these techniques involve the coastal oceans, there may be opportunities to remediate acidified ocean conditions in some instances. However, these marine CDR techniques are in their infancy and will require additional study to limit the risks associated with deployment, despite the potential benefits of atmospheric carbon sequestration and OA mitigation. It will also be essential to consider the ethical and climate justice ramifications of these techniques, especially regarding Arctic communities and peoples (Cassotta 2021).
References
AMAP, 2018: AMAP Assessment 2018: Arctic Ocean Acidification. Arctic Monitoring and Assessment Programme (AMAP), Tromsø, Norway. Vi+187 pp.
Anglada-Ortiz, G., K. Zamelczyk, J. Meilland, P. Ziveri, M. Chierici, A. Fransson, and T. L. Rasmussen, 2021: Planktic foraminiferal and pteropod contributions to carbon dynamics in the Arctic Ocean (North Svalbard Margin). Front. Mar. Sci., 8, 661158, https://doi.org/10.3389/fmars.2021.661158.
Ardyna, M., and K. R. Arrigo, 2020: Phytoplankton dynamics in a changing Arctic Ocean. Nat. Climate Change, 10(10), 892-903, https://doi.org/10.1038/s41558-020-0905-y.
Beaupré-Laperrière, A., A. Mucci, and H. Thomas, 2020: The recent state and variability of the carbonate system of the Canadian Arctic Archipelago and adjacent basins in the context of ocean acidification. Biogeosciences, 17, 3923-3942, https://doi.org/10.5194/bg-17-3923-2020.
Bednaršek, N., and Coauthors, 2021: Integrated assessment of ocean acidification risks to pteropods in the northern high latitudes: Regional comparison of exposure, sensitivity and adaptive capacity. Front. Mar. Sci., 8, 671497, https://doi.org/10.3389/fmars.2021.671497.
Cassotta, S., 2021: Ocean acidification in the Arctic in a multi-regulatory, climate-justice perspective. Front. Climate, 3, 713644. https://doi.org/10.3389/fclim.2021.713644.
Chierici, M., M. Vernet, A. Fransson, and K. Y. Børsheim, 2019: Net community production and carbon exchange from winter to summer in the Atlantic Inflow to the Arctic Ocean. Front. Mar. Sci., 6, 528, https://doi.org/10.3389/fmars.2019.00528.
Duarte, C. M., A. B. Rodriguez-Navarro, A. Delgado-Huertas, and D. Krause-Jensen, 2020: Dense mytilus beds along freshwater-influenced Greenland shores: Resistance to corrosive waters under high food supply. Estuar. Coast., 43(2), 387-395, https://doi.org/10.1007/s12237-019-00682-3.
Goethel, C. L., J. M. Grebmeier, L. W. Cooper, and T. J. Miller, 2017: Implications of ocean acidification in the Pacific Arctic: experimental responses of three Arctic bivalves to decreased pH and food availability. Deep-Sea Res. Pt. II, 144, 112-124, https://doi.org/10.1016/j.dsr2.2017.08.013.
Hänsel, M. C., J. O. Schmidt, M. H. Stiasny, M. T. Stöven, R. Voss, and M. F. Quaas, 2020: Ocean warming and acidification may drag down the commercial Arctic cod fishery by 2100. PLoS One, 15(4), e0231589, https://doi.org/10.1371/journal.pone.0231589.
IPCC, 2021: Climate Change 2021: The Physical Science Basis. Contribution of Working Group I to the Sixth Assessment Report of the Intergovernmental Panel on Climate Change [V. Masson-Delmotte, and coeditors, (eds.)]. Cambridge University Press.
Jones, E. M., M. Chierici, S. Menze, A. Fransson, R. B. Ingvaldsen, and H. H. Lødemel, 2021: Ocean acidification state variability of the Atlantic Arctic Ocean around northern Svalbard. Prog. Oceanogr., 199, 102708, https://doi.org/10.1016/j.pocean.2021.102708.
Mortenson, E., N. Steiner, A. H. Monahan, H. Hayashida, T. Sou, and A. Shao, 2020: Modeled impacts of sea ice exchange processes on Arctic Ocean carbon uptake and acidification, 1980-2015. J. Geophys. Res.-Oceans, 125(7), e2019JC015782, https://doi.org/10.1029/2019JC015782.
Niemi, A., N. Bednaršek, C. Michel, R. A. Feely, W. Williams, K. Azetsu-Scott, W. Walkusz, and J. D. Reist, 2021: Biological impact of ocean acidification in the Canadian Arctic: Widespread severe pteropod shell dissolution in Amundsen Gulf. Front. Mar. Sci., 8, 600184, https://doi.org/10.3389/fmars.2021.600184.
Pilcher, D. J., D. M. Naiman, J. N. Cross, A. J. Hermann, S. A. Siedlecki, G. A. Gibson, and J. T. Mathis, 2019: Modeled effect of coastal biogeochemical processes, climate variability, and ocean acidification on aragonite saturation state in the Bering Sea. Front. Mar. Sci., 5, 508, https://doi.org/10.3389/fmars.2018.00508.
Qi, D., and Coauthors, 2017: Increase in acidifying water in the western Arctic Ocean. Nat. Climate Change, 7, 195-199, https://doi.org/10.1038/NCLIMATE3228.
Qi, D., and Coauthors, 2020. Coastal acidification induced by biogeochemical processes driven by sea-ice melt in the western Arctic Ocean. Polar Sci., 23, 100504, https://doi.org/10.1016/j.polar.2020.100504.
Steiner, N. S., and Coauthors, 2019: Impacts of changing ocean-sea ice system on the key forage fish Arctic Cod (Boreogadus saida) and subsistence fisheries in the western Canadian Arctic—evaluating linked climate, ecosystem, and economic (CEE) models. Front. Mar. Sci., 6, 179, https://doi.org/10.3389/fmars.2019.00179.
Sugie, K., A. Fujiwara, S. Nishino, S. Kameyama, and N. Harada, 2020: Impacts of temperature, CO2, and salinity on phytoplankton community composition in the western Arctic Ocean. Front. Mar. Sci., 6, 821, https://doi.org/10.3389/fmars.2019.00821.
Tai, T. C., U. R. Sumaila, and W. W. L. Cheung, 2021: Ocean acidification amplifies multi-stressor impacts on global marine invertebrate fisheries. Front. Mar. Sci., 8, 596644. https://doi.org/10.3389/fmars.2021.596644.
Terhaar, J., L. Kwiatkowski, and L. Bopp, 2020: Emergent constraint on Arctic Ocean acidification in the twenty-first century. Nature, 582, 379-383, https://doi.org/10.1038/s41586-020-2360-3.
Torstensson, A., A. R. Margolin, G. M. Showalter, W. O. Smith Jr., E. H. Shadwick, S. D. Carpenter, F. Bolinesi, and J. W. Deming, 2021: Sea-ice microbial communities in the Central Arctic Ocean: Limited responses to short-term pCO2 perturbations. Limnol. Oceanogr., 66(S1), S383-S400, https://doi.org/10.1002/lno.11690.
Ulfsbo, A., E. M. Jones, N. Casacuberta, M. Korhonen, B. Rabe, M. Karcher, and S. M. A. C. van Heuven, 2018: Rapid changes in anthropogenic carbon storage and ocean acidification in the intermediate layers of the Eurasian Arctic Ocean: 1996-2015. Global Biogeochem. Cycles, 32(9), 1254-1275, https://doi.org/10.1029/2017GB005738.
Zhang, Y., M. Yamamoto-Kawai, W. J. Williams, 2020: Two decades of ocean acidification in the surface waters of the Beaufort Gyre, Arctic Ocean: Effects of sea ice melt and retreat from 1997-2016. Geophys. Res. Lett., 47(3), e60119, https://doi.org/10.1029/2019GL086421.
November 23, 2021