G. J. Wolken1,2, A. K. Liljedahl3, M. Brubaker4, J. A. Coe5, G. Fiske3, H. Hvidtfeldt Christiansen6, M. Jacquemart7,8, B. M. Jones9, A. Kääb10, F. Løvholt11, S. Natali3, A. C. A. Rudy12, and D. Streletskiy13
1International Arctic Research Center, University of Alaska Fairbanks, Fairbanks, AK, USA
2Alaska Division of Geological & Geophysical Surveys, Department of Natural Resources, Fairbanks, AK, USA
3Woodwell Climate Research Center, Falmouth, MA, USA
4Alaska Native Tribal Health Consortium, Anchorage, AK, USA
5Geologic Hazards Science Center, USGS, Golden, CO, USA
6The University Centre in Svalbard, UNIS, Svalbard, Norway
7Laboratory of Hydraulics, Hydrology, and Glaciology (VAW), Department of Civil, Environmental and Geomatic Engineering, ETH Zurich, Zurich, Switzerland
8Swiss Federal Institute of Forest, Snow, and Landscape Research (WSL), Birmensdorf, Switzerland
9Water and Environmental Research Center, Institute of Northern Engineering, University of Alaska Fairbanks, Fairbanks, AK, USA
10Department of Geosciences, University of Oslo, Oslo, Norway
11Geohazards and Dynamics, NGI, Oslo, Norway
12Northwest Territories Geological Survey, Government of the Northwest Territories, Yellowknife, NT, Canada
13Department of Geography, The George Washington University, Washington, DC, USA
Highlights
- Retreating glaciers and thawing permafrost are causing local- to regional-scale hazards that threaten lives and livelihoods, infrastructure, sustainable resource development, and national security.
- Permafrost hazards are gradually impacting people across the Arctic, while glacier/permafrost hazard cascades are abrupt, more localized, and most life threatening.
- Broad-scale hazard identification and assessment across the Arctic are needed to better inform stakeholder decision making.
Introduction
Air temperature increases in the Arctic over the last two decades have been more than twice the global average, prompting an acceleration in glacier mass loss and permafrost degradation (IPCC 2019; Hugonnet et al. 2021; Smith et al. 2021). Beyond the global implications of these rapid changes (e.g., carbon release and sea level rise), the emergence and increase in cryosphere hazards threaten national security (e.g., military infrastructure and population displacement) and the lives of Arctic residents across local to regional scales. Here, we focus on hazards related to glaciers and permafrost and define hazard as the potential occurrence of a natural physical process that may adversely impact human or ecological systems (IPCC 2019).
Observations of glacier and permafrost hazards
About five million people live in the Northern Hemisphere permafrost region, which includes glaciers, and within this region, glacier and permafrost hazards are affecting lives, infrastructure, and ecosystem services (Ramage et al. 2021; Fig. 1). Recent degradation of glaciers and permafrost in the Arctic are leading to emerging biogeochemical threats that have the potential to disrupt ecosystem function and endanger human health (Miner et al. 2021). Thawing of ice-rich permafrost can cause ground subsidence with negative implications for infrastructure, ecosystems, and human lives and livelihoods (Suter et al. 2019; Gibson et al. 2021), while even a warming of permafrost can cause a reduction in its bearing capacity, impacting its ability to support structures (Streletskiy et al. 2012). This is especially apparent in the Russian Arctic where there are centers of high population and industrial economic activity in permafrost zones that act as foci of human-induced permafrost degradation, exacerbating climatically driven changes in the permafrost system (Vasiliev et al. 2020). For example, the recent oil tank collapse in Norilsk, Russia that resulted in the release of 21,000 cubic meters of diesel oil was at least partially attributed to the extremely warm conditions of 2020 in addition to a long-term warming trend in the region (Rajendran et al. 2021). Thawing of cold continuous permafrost in Point Lay and Wainwright, Alaska, caused a complete water system failure for multiple homes and buildings, including the health clinic (Cameron and Romanovsky 2021). As recently as 2009, the borough assumed the risk of thawing as “low” because the permafrost was classified as “continuous” and thought to be cold and stable (Cameron and Romanovsky 2021), yet permafrost degradation through the process of thermal erosion of ground ice also drained the community’s drinking water source lake (Fig. 2; Dobbin 2016). Mountain permafrost degradation can also increase the likelihood of landslides (Hock et al. 2019). For example, a rock avalanche endangered two farms and destroyed a considerable amount of livestock pastures in Signaldalen, northern Norway (Frauenfelder et al. 2018). Field observations showed that the upper limit of the failure corresponded to the lower altitudinal limit of permafrost. The combination of gradual long-term warming and record-high mean near-surface temperatures caused the rock avalanche.
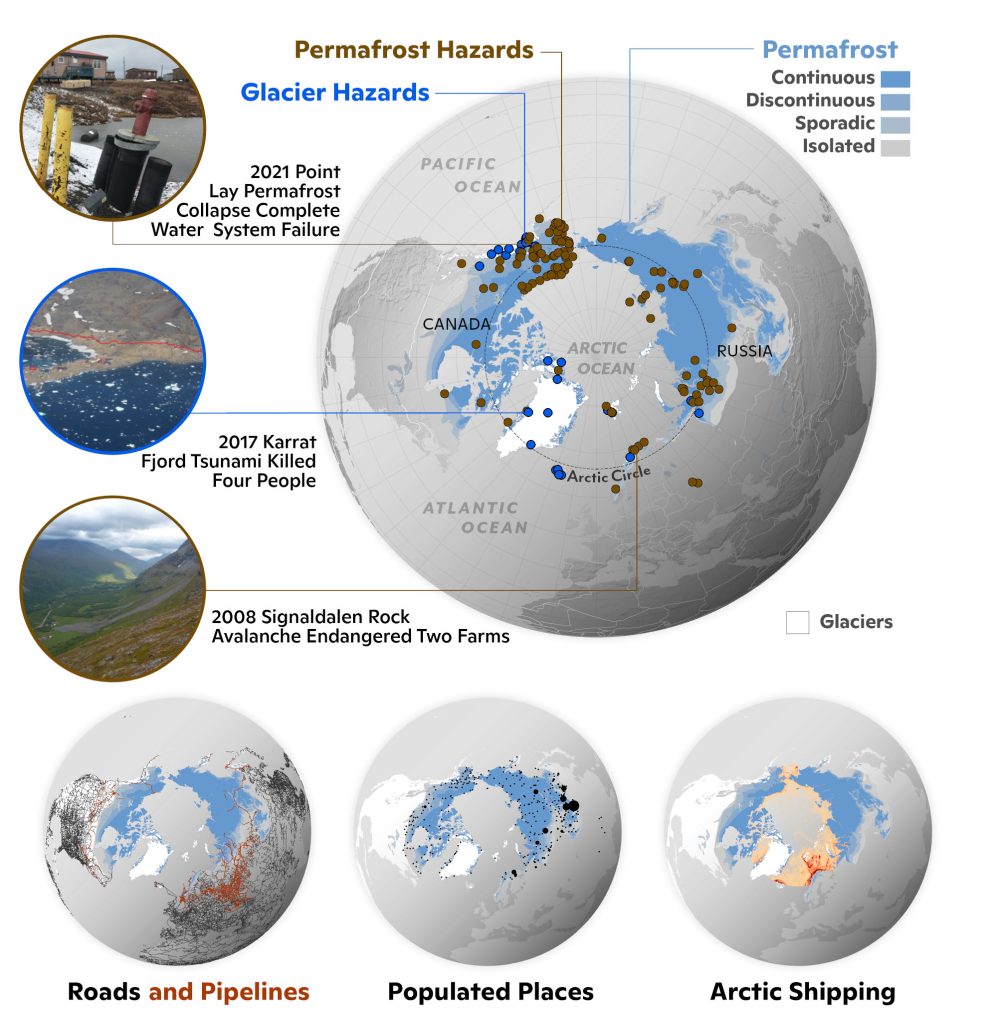
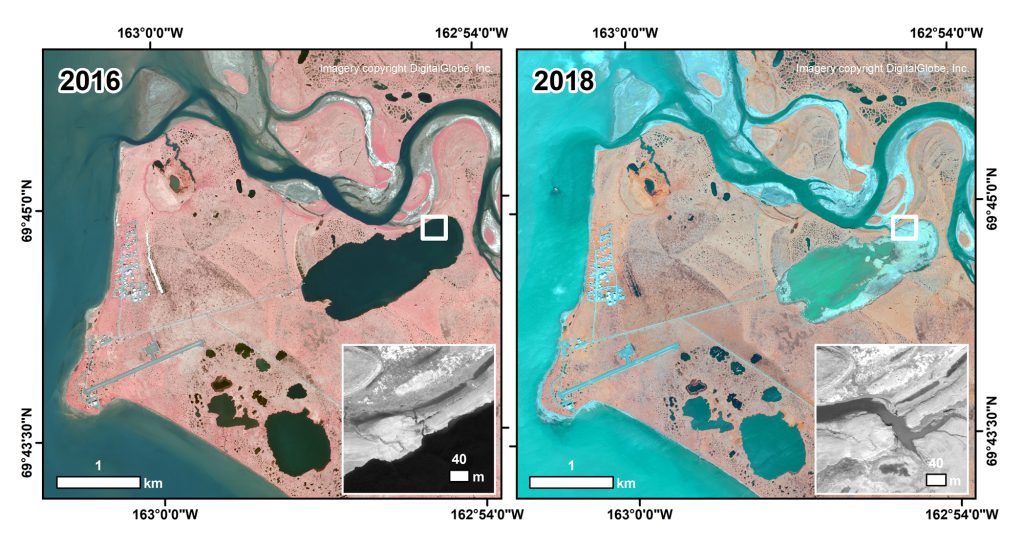
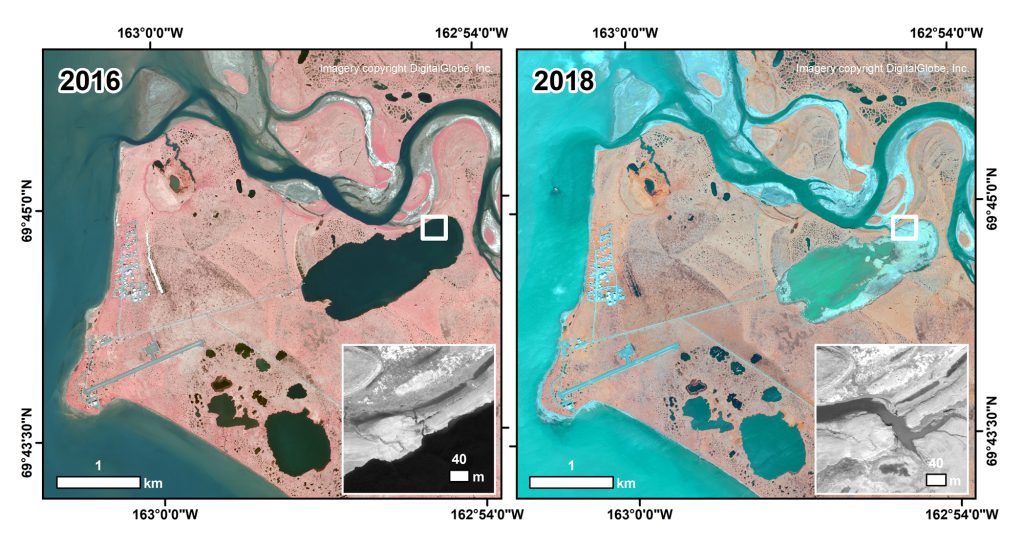
Glacier retreat exposes over-steepened slopes that are prone to destabilization and, if in the presence of deep water, can cause landslide-generated tsunamis (Dai et al. 2020). The Karrat Fjord rock avalanche in 2017 generated a tsunami that killed four people in the village of Nuugaatsiaq, Greenland (Gauthier et al. 2018). Persistent unstable slope hazards keep residents of Nuugaatsiaq from returning home, while similar hazards (Barry Arm fjord) in northwest Prince William Sound, Alaska, have prompted advisories of unsafe travel and possible tsunami inundation. Unstable glacierized mountain regions in southeast Alaska have produced landslides that have generated the tallest tsunamis in the world (Higman et al. 2018), and despite the remote location, these hazards threaten communities, marine traffic, and infrastructure including major communication cables of importance to national security. Glacial lake outburst floods (GLOFs or Jökulhlaups) are abrupt releases of water from glacierized catchments that can significantly endanger downstream communities and infrastructure as well (Kienholz et al. 2020). In response to regular GLOFs from the Vatnajökull ice cap, the government of Iceland has developed a warning system to give local residents time to evacuate and, considering the regularity of GLOF occurrences, knowledge of where to expect flooding. Surging glaciers, where the glacier moves 10-100 times faster than typical, can also be hazardous and have made travel routes impassable in Svalbard, Norway.
A cascade of glacier and permafrost hazards
Glacier and permafrost hazards occur along a spectrum of spatial, temporal, and intensity scales. The largest disasters in terms of reach, damage, and lives lost that involve glaciers and permafrost occur typically through a combination or chain of processes, each potentially representing a hazard by itself. For instance, slope failures and glacier detachments can trigger cascading hazards, especially when slope failures enter water bodies and cause outburst floods, debris flows, and tsunamis (Haeberli et al. 2017; Higman et al. 2018; Jacquemart et al. 2020). These cascading hazards can present a risk to people and infrastructure at great distances (102 km) from the initiating slope failure, and changes in climate can shift hazard zones and scales. For instance, as calving glaciers retreat, larger water bodies are often exposed, increasing the potential for rock avalanches to enter the water and generate devastating displacement waves, which increase the reach and intensity of the hazard.
Drivers of glacier and permafrost hazards
Thawing of permafrost and melting of land ice that formed and was retained over millennia are altering the Arctic landscape baseline condition, with extreme weather events further amplifying the potential for glacier and permafrost hazards. Permafrost temperatures have increased across the circumpolar region since the 1980s (Smith et al. 2021), and permafrost degradation has been documented across much of the Arctic (Liljedahl et al. 2016; Vasiliev et al. 2020). The onset of ice-rich permafrost degradation has been linked to long-term gradual warming combined with extreme events such as unusually warm summers and deep snow cover (Farquharson et al. 2019). A period of unusually warm air temperatures between 2012 and 2016 in southeast Alaska coincided with an increase in rock-avalanche activity and size (Bessette-Kirton and Coe 2020). The influence of degrading permafrost on landslide occurrence is increasingly recognized throughout the Arctic, including Norway (Hilger et al. 2021), Iceland (Sæmundsson et al. 2018), Russia (Leibman et al. 2015), and Canada (Lewkowicz and Way 2019).
Observations of extreme precipitation, such as more intense and prolonged rainfall (e.g., atmospheric rivers), appear to be increasing (Francis et al. 2021). While extreme precipitation events alone can trigger deadly debris flows, such as in Sitka (August 2015) and Haines, Alaska (December 2020), increased rainfall, combined with warming air temperatures, is also documented to drive landslide development in ice-rich permafrost terrain (Kokelj et al. 2015). Similarly, the combination of increased rainfall, unusual warmth that increases glacial ice melt, more crevasses, and retreat and steepening of a glacier appears to encourage glacier surges (Sevestre et al. 2018).
Mountain permafrost is the least monitored permafrost type in the Arctic, and processes linking glaciers and permafrost are poorly understood. Paleorecords and newly discovered processes, however, point to conditions that elevate hazard potential in glacier and permafrost areas. In Norway, the frequency of postglacial landslide activity was dominated by events in the beginning of the Holocene when and where ice sheet retreat was most rapid (Bellwald et al. 2016). Newly discovered processes, e.g., the release of entire glacier tongues from their beds, have been documented in the Arctic, but more data are needed to understand the conditions that favor devastating mass flows of water, ice, and debris (Jacquemart et al. 2020).
Outlook and needs
As the Arctic landscape continues to respond to warming conditions, attention to glacier and permafrost hazards will become increasingly important. The clustering of landslide and glacier detachment events in the immediate aftermath of warming episodes, such as following the last deglaciation, clearly flags the likelihood of increased hazard due to global warming. The recent major landslide tsunami events in Paatuut, Greenland 2000 (Dahl-Jensen et al. 2004), Karrat Fjord, Greenland 2017 (Svennevig et al. 2020), and Taan Fjord, Alaska 2017 (Higman et al. 2018) serve as recent stand-out examples of glacier and permafrost hazards in the Arctic. These events highlight the need for broad-scale hazard identification and assessment to improve our knowledge of glacier and permafrost hazards and better inform stakeholder decision making.
The following are challenges and needs regarding Arctic glacier and permafrost hazards:
- A core need is more extensive baseline data (e.g., ground ice content), via remote sensing, field observations, and community science, to identify hazards and evaluate potential landscape change for adaptation and mitigation planning;
- Existing permafrost and glacier monitoring networks could assist in identifying areas of concern, while also guiding the formation of new monitoring networks;
- Long-term observation of mountain permafrost in the Arctic and refined understanding of how permafrost degradation and glacier retreat processes impact slope stability are needed; and
- Future efforts to address glacier and permafrost hazards in the Arctic will require implementation of a co-production approach (e.g., community members, scientists, and engineers) to find effective adaptation and preparedness options (i.e., early warning systems) to enhance resilience.
Acknowledgments
We thank Matt Thomas, Twila Moon, Rick Thoman, Rex Baum, Brian Shiro, Janet Slate, and three anonymous reviewers for their constructive reviews of this article. G. J. Wolken received partial support from the Alaska Climate Adaptation Science Center. B. M. Jones was supported by the US National Science Foundation under grants OISE-1927553 and OPP-1806213. Any use of trade, firm, or product names is for descriptive purposes only and does not imply endorsement by the U.S. Government.
References
Arp, C. D., B. M. Jones, K. M. Hinkel, D. L. Kane, M. S. Whitman, and R. Kemnitz, 2020: Recurring outburst floods from drained lakes: An emerging Arctic hazard. Front. Ecol. Environ., 18(7), 384-390, https://doi.org/10.1002/fee.2175.
Bellwald, B., B. O. Hjelstuen, H. P. Sejrup, and H. Haflidason, 2016: Postglacial mass movements and depositional environments in a high-latitude fjord system—Hardangerfjorden, Western Norway. Mar. Geol., 379, 157-175, https://doi.org/10.1016/j.margeo.2016.06.002.
Berkman, P. A, G. Fiske, and D. Lorenzini, 2020: Baseline of next-generation Arctic marine shipping assessments – Oldest continuous pan-Arctic satellite Automatic Identification System (AIS) data record of maritime ship traffic, 2009-2016. Arctic Data Center, https://doi.org/10.18739/A2TD9N89Z.
Bessette-Kirton, E. K., and J. A. Coe, 2020: A 36-year record of rock avalanches in the St. Elias Mountains of southeast Alaska, with implications for future hazards. Front. Earth Sci., 8, 293, https://doi.org/10.3389/feart.2020.00293.
Brown, J., O. J. Ferrians Jr., J. A. Heginbottom, and E. S. Melnikov, 1997: Circum-Arctic Map of Permafrost and Ground-Ice Conditions. Circum-Pacific Map CP-45, 1:10,000,000-Scale. Washington, DC, U.S. Geological Survey in Cooperation with the Circum-Pacific Council for Energy and Mineral Resources. 1 sheet, https://doi.org/10.3133/cp45.
Cameron, R., and V. Romanovsky, 2021: Thawing permafrost and subsidence causing on-going water system challenges. LEO Network (leonetwork.org). Accessed 13 September 2021.
Dahl-Jensen, T., and Coauthors, 2004: Landslide and tsunami 21 November 2000 in Paatuut, West Greenland. Nat. Hazards, 31, 277-287, https://doi.org/10.1023/B:NHAZ.0000020264.70048.95.
Dai, C., and Coauthors, 2020: Detection and assessment of a large and potentially tsunamigenic periglacial landslide in Barry Arm, Alaska. Geophys. Res. Lett., 47(22), e2020GL089800, https://doi.org/10.1029/2020GL089800.
Dobbin, P., 2016: DEC looks into whether drums in village’s drinking water lake pose any hazards. Alaska’s News Source (9 Aug 2016), https://www.alaskasnewssource.com/content/news/Officials-try-to-determine-if-Point-Lays-drinking-water-is-safe-or-contaminated-389669191.html, Accessed 13 Sep 2021.
Farquharson, L. M., V. E. Romanovsky, W. L. Cable, D. A. Walker, S. V. Kokelj, and D. Nicolsky, 2019: Climate change drives widespread and rapid thermokarst development in very cold permafrost in the Canadian High Arctic. Geophys. Res. Lett., 46(12), 6681-6689, https://doi.org/10.1029/2019GL082187.
Francis, J. A., N. Skific, S. J. Vavrus, and J. Cohen, 2021: Measuring “weather whiplash” events in North America: A new large-scale regime approach. Preprint ESSOAr, https://doi.org/10.1002/essoar.10507297.1.
Frauenfelder, R., K. Isaksen, M. J. Lato, and J. Noetzli, 2018: Ground thermal and geomechanical conditions in a permafrost-affected high-latitude rock avalanche site (Polvartinden, northern Norway). Cryosphere, 12(4), 1531-1550, https://doi.org/10.5194/tc-12-1531-2018.
Gauthier, D., S. A. Anderson, H. M. Fritz, and T. Giachetti, 2018: Karrat Fjord (Greenland) tsunamigenic landslide of 17 June 2017: Initial 3D observations. Landslides, 15(2), 327-332, https://doi.org/10.1007/s10346-017-0926-4.
Gibson, C. M., T. Brinkman, H. Cold, D. Brown, and M. Turetsky, 2021: Identifying increasing risks of hazards for northern land-users caused by permafrost thaw: Integrating scientific and community-based research approaches. Environ. Res. Lett., 16(6), 064047, https://doi.org/10.1088/1748-9326/abfc79.
Haeberli, W., Y. Schaub, and C. Huggel 2017: Increasing risks related to landslides from degrading permafrost into new lakes in de-glaciating mountain ranges. Geomorphology, 293, 405-417, https://doi.org/10.1016/j.geomorph.2016.02.009.
Higman, B., and Coauthors, 2018: The 2015 landslide and tsunami in Taan Fiord, Alaska. Sci. Rep., 8, 12993, https://doi.org/10.1038/s41598-018-30475-w.
Hilger, P., R. L. Hermanns, J. Czekirda, K. S. Myhra, J. S. Gosse, and B. Etzelmüller, 2021: Permafrost as a first order control on long-term rock-slope deformation in (Sub-) Arctic Norway. Quat. Sci. Rev., 251, 106718, https://doi.org/10.1016/j.quascirev.2020.106718.
Hock, R., and Coauthors, 2019: High Mountain Areas, in: IPCC Special Report on the Ocean and Cryosphere in a Changing Climate (SROCC) [H. -O. Pörtner, and Coauthors (eds.)], IPCC, Geneva. In press.
Hugonnet, R., and Coauthors, 2021: Accelerated global glacier mass loss in the early twenty-first century. Nature, 592, 726-731, https://doi.org/10.1038/s41586-021-03436-z.
IPCC, 2019: IPCC Special Report on the Ocean and Cryosphere in a Changing Climate [H. -O. Pörtner, and Coauthors (eds.)]. In press.
Jacquemart, M., M. Loso, M. Leopold, E. Welty, E. Berthier, J. S. Hansen, J. Sykes, and K. Tiampo, 2020: What drives large-scale glacier detachments? Insights from Flat Creek glacier, St. Elias Mountains, Alaska. Geology, 48(7), 703-707, https://doi.org/10.1130/G47211.1.
Kienholz, C., and Coauthors, 2020: Deglacierization of a marginal basin and implications for outburst floods, Mendenhall Glacier, Alaska. Front. Earth Sci., 8, 137, https://doi.org/10.3389/feart.2020.00137.
Kokelj, S. V., J. Tunnicliffe, D. Lacelle, T. C. Lantz, K. S. Chin, and R. Fraser, 2015: Increased precipitation drives mega slump development and destabilization of ice-rich permafrost terrain, northwestern Canada. Global Planet Change, 129, 56-68, https://doi.org/10.1016/j.gloplacha.2015.02.008.
Leibman, M. O., A. V. Khomutov, A. A. Gubarkov, Y. A. Dvornikov, and D. R. Mullanurov, 2015: The research station “Vaskiny Dachi”, central Yamal, West Siberia, Russia—A review of 25 years of permafrost studies. Fennia, 193(1), 3-30, https://doi.org/10.11143/45201.
Lewkowicz, A. G., and R. G. Way, 2019: Extremes of summer climate trigger thousands of thermokarst landslides in a High Arctic environment. Nat. Commun., 10, 1329, https://doi.org/10.1038/s41467-019-09314-7.
Liljedahl, A. K., and Coauthors, 2016: Pan-Arctic ice-wedge degradation in warming permafrost and its influence on tundra hydrology. Nat. Geosci., 9, 312-318, https://doi.org/10.1038/ngeo2674.
Miner, K. R., J. D’Andrilli, R. Mackelprang, A. Edwards, M. J. Malaska, M. P. Waldrop, and C. E. Miller, 2021: Emergent biogeochemical risks from Arctic permafrost degradation. Nat. Climate Change, 11, 809-819, https://doi.org/10.1038/s41558-021-01162-y.
Nitze, I., S. W. Cooley, C. R. Duguay, B. M. Jones, and G. Grosse, 2020: The catastrophic thermokarst lake drainage events of 2018 in northwestern Alaska: Fast-forward into the future. Cryosphere, 14, 4279-4297, https://doi.org/10.5194/tc-14-4279-2020.
Rajendran, S., F. N. Sadooni, H. A. -S. Al-Kuwari, A. Oleg, H. Govil, S. Nasir, and P. Vethamony, 2021: Monitoring oil spill in Norilsk, Russia using satellite data. Sci. Rep., 11, 3817, https://doi.org/10.1038/s41598-021-83260-7.
Ramage, J., L. Jungsberg, S. Wang, S. Westermann, H. Lantuit, and T. Heleniak, 2021: Population living on permafrost in the Arctic. Popul. Environ., 43, 22-38, https://doi.org/10.1007/s11111-020-00370-6.
Sæmundsson, Þ., C. Morino, J. K. Helgason, S. J. Conway, and H. G. Pétursson, 2018: The triggering factors of the Móafellshyrna debris slide in northern Iceland: Intense precipitation, earthquake activity and thawing of mountain permafrost. Sci. Total. Environ., 621, 1163-1175, https://doi.org/10.1016/j.scitotenv.2017.10.111.
Sevestre, H., D. I. Benn, A. Luckman, C. Nuth, J. Kohler, K. Lindbäck, and R. Pettersson, 2018: Tidewater glacier surges initiated at the terminus. J. Geophys. Res.-Earth Surf., 123(5), 1035-1051, https://doi.org/10.1029/2017JF004358.
Smith, S. L., and Coauthors, 2021: [Arctic] Permafrost [in “State of the Climate in 2020”]. Bull. Amer. Meteor., 102(8), S293-S297, https://doi.org/10.1175/BAMS-D-21-0086.1.
Streletskiy, D. A., N. I. Shiklomanov, and F. E. Nelson, 2012: Permafrost, infrastructure and climate change: A GIS-based landscape approach to geotechnical modeling. Arct. Antarct. Alp. Res., 44(3), 368-380, https://doi.org/10.1657/1938-4246-44.3.368.
Suter, L., D. Streletskiy, and N. Shiklomanov. 2019: Assessment of the costs of climate change impacts on critical infrastructure in the circumpolar Arctic. Polar Geogr., 42(4), 267-286, https://doi.org/10.1080/1088937X.2019.1686082.
Svennevig, K., T. Dahl-Jensen, M. Keiding, J. P. Merryman Boncori, T. B. Larsen, S. Salehi, A. Munck Solgaard, and P. H. Voss, 2020: Evolution of events before and after the 17 June 2017 rock avalanche at Karrat Fjord, West Greenland—A multidisciplinary approach to detecting and locating unstable rock slopes in a remote Arctic area. Earth Surf. Dyn., 8(4), 1021-1038, https://doi.org/10.5194/esurf-8-1021-2020.
Vasiliev, A. A., D. S. Drozdov, A. G. Gravis, G. V. Malkova, K. E. Nyland, and D. A. Streletskiy, 2020: Permafrost degradation in the Western Russian Arctic. Environ. Res. Lett., 15(4), 045001, https://doi.org/10.1088/1748-9326/ab6f12.
January 5, 2022