A. York1, U. Bhatt2, R. Thoman3, R. Ziel1
1Alaska Fire Science Consortium, International Arctic Research Center, University of Alaska, Fairbanks, AK, USA
2Geophysical Institute, University of Alaska, Fairbanks, AK, USA
3National Weather Service, Alaska Region, Anchorage, AK, USA
Highlights
- High latitude fire regimes appear to be responding rapidly to environmental changes associated with a warming climate; although highly variable, area burned has increased over the past several decades in much of Boreal North America.
- Most acreage burned in high latitude systems occurs during sporadic periods when lightning ignitions coincide with warm and dry weather that cures vegetation and elevates fire danger.
- Under a range of climate change scenarios, analyses using multiple approaches project significant increases (up to four-fold) in area burned in high latitude ecosystems by the end of the 21st century.
Despite the low annual temperatures and short growing seasons characteristic of northern ecosystems, wildland fire affects both boreal forest (the broad band of mostly coniferous trees that generally stretches across the area north of the July 13° C isotherm in North America and Eurasia, also known as Taiga) and adjacent tundra regions. In fact, fire is the dominant ecological disturbance in boreal forest, the world’s largest terrestrial biome. Fire disturbance affects these high latitude systems at multiple scales, including direct release of carbon through combustion (Kasischke et al., 2000) and interactions with vegetation succession (Mann et al., 2012; Johnstone et al., 2010), biogeochemical cycles (Bond-Lamberty et al., 2007), energy balance (Rogers et al., 2015), and hydrology (Liu et al., 2005). About 35% of global soil carbon is stored in tundra and boreal systems (Scharlemann et al., 2014) that are potentially vulnerable to fire disturbance (Turetsky et al., 2015). This brief report summarizes evidence from Alaska and Canada on variability and trends in fire disturbance in high latitudes and outlines how short-term fire weather conditions in these regions influence area burned.
Beyond immediate threats to lives and property, wildland fire has multiple impacts on northern environments and residents. Smoke can be widespread and compromise health, particularly in vulnerable populations (Reid et al., 2016). Wildfire smoke can also limit visibility and constrain aviation operations, including those supporting fire suppression and detection. Black carbon, derived from wildfires, is known to travel considerable distances and accelerate surface melting and thawing of snow and ice (Thomas et al., 2017). Removal of the surface organic layer by combustion has been shown to initiate the development of thermokarst in both boreal and tundra soils, which are rich in permafrost (Jorgenson et al., 2010; Jones et al., 2015).
Climate is a dominant control of fire activity in both boreal and tundra ecosystems. The relationship between climate and fire is strongly nonlinear, with the likelihood of fire occurrence within a 30-year period much higher where mean July temperatures exceed 13.4° C (56° F) (Young et al., 2017). High latitude fire regimes appear to be responding rapidly to recent environmental changes associated with the warming climate. Although highly variable, area burned has increased over the past several decades in much of boreal North America (Kasischke and Turetsky, 2006; Gillett et al., 2004). Since the early 1960s, the number of individual fire events and the size of those events has increased, contributing to more frequent large fire years in northwestern North America (Kasischke and Turetsky, 2006). Figure 1 shows annual area burned per year in Alaska (a) and Northwest Territories (b) since 1980, including both boreal and tundra regions.
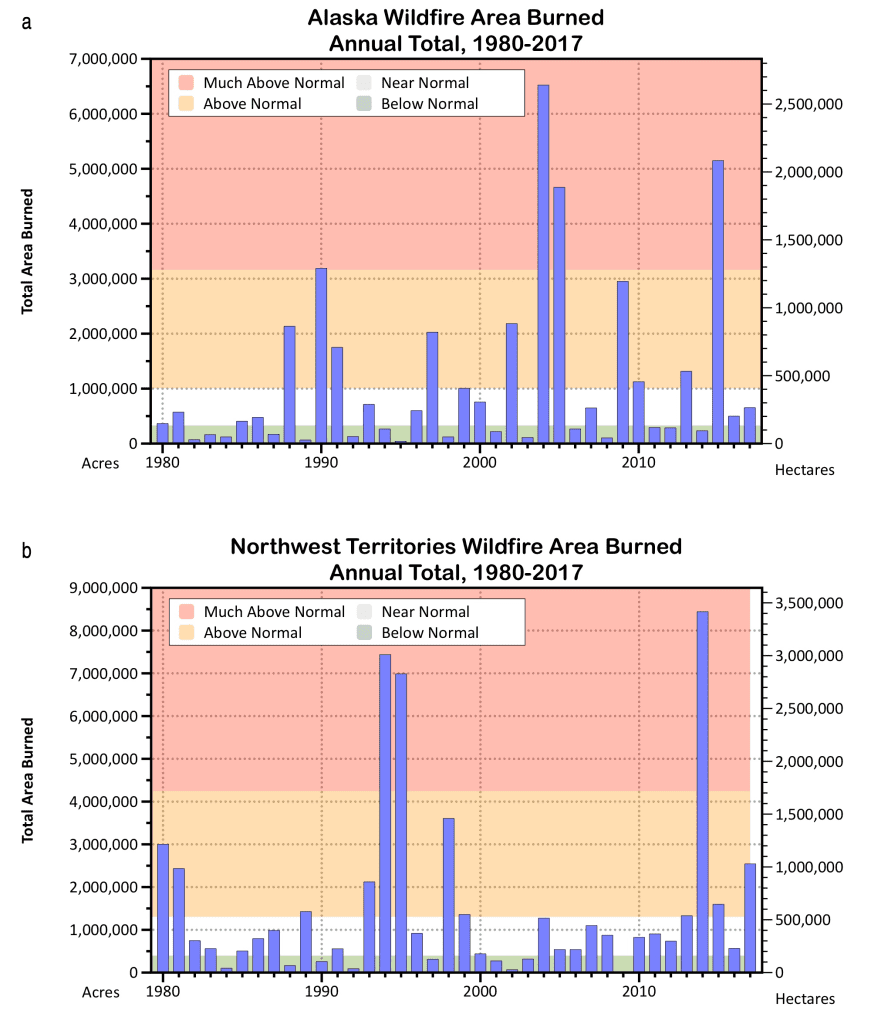
Recent large fire seasons in high latitudes include 2014 in the Northwest Territories, where 385 fires burned 8.4 million acres, and 2015 in Alaska, where 766 fires burned 5.1 million acres (Figs. 1 & 2)—more than half the total acreage burned in the US (NWT, 2015; AICC, 2015). Multiple northern communities have been threatened or damaged by recent wildfires, notably Fort McMurray, Alberta, where 88,000 people were evacuated and 2400 structures were destroyed in May 2016. Examples of recent significant tundra fires include the 2007 Anaktuvuk River Fire, the largest and longest-burning fire known to have occurred on the North Slope of Alaska (256,000 acres), which initiated widespread thermokarst development (Jones et al., 2015). An unusually large tundra fire in western Greenland in 2017 received considerable media attention.
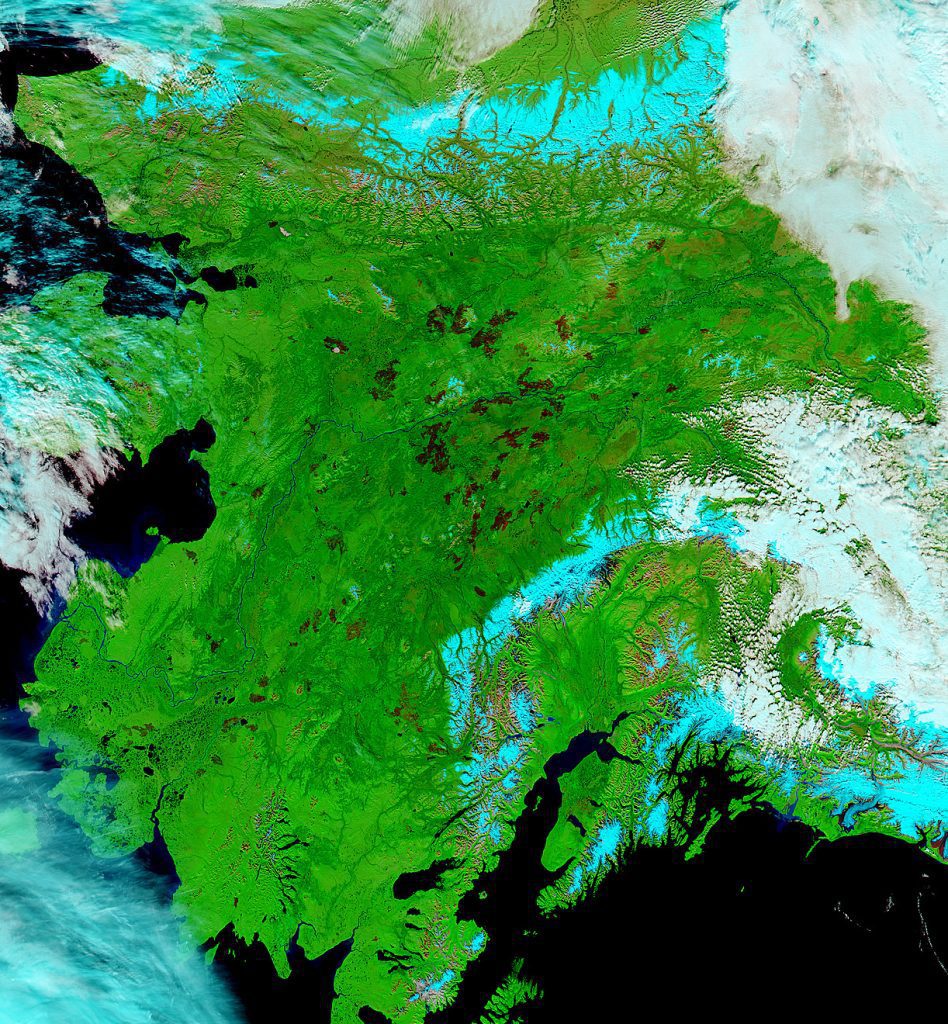
Large fire events such as these require the confluence of receptive fuels that will promote fire growth once ignited, periods of warm and dry weather conditions, and a source of ignition—most commonly, convective thunderstorms that produce lightning ignitions. High latitude ecosystems are characterized by unique fuels—in particular, fast-drying beds of mosses, lichens, resinous shrubs, and accumulated organic material (duff) that underlie dense, highly flammable conifers. These understory fuels cure rapidly during warm, dry periods with long daylight hours in June and July. Consequently, extended periods of drought are not required to increase fire danger to extreme levels in these systems.
Lightning has historically been responsible for the majority of acres burned in high latitudes, as lightning-ignited fires tend to occur in more remote locations and thus are subject to lower levels of suppression than human-started incidents. Veraverbeke et al. (2017) recently showed that lightning ignitions have increased in boreal North America since 1975 and largely drove the extreme 2014 Northwest Territories and 2015 Alaska fire seasons. In addition, Partain et al. (2016) found that human-induced climate change, manifested as a combination of high surface temperatures, low relative humidity, and low precipitation, increased the likelihood of the extremely dry fuel conditions seen in Alaska in 2015 by 34-60%.
Most acreage burned in high latitude systems occurs during sporadic periods of high fire activity; 50% of the acreage burned in Alaska from 2002 to 2010 was consumed in just 36 days (Barrett et al., 2016). Figure 3 shows cumulative acres burned in the four largest fire seasons in Alaska since 1990 (from Fig. 1) and illustrates the varying trajectories of each season. Some seasons show periods of rapid growth during unusually warm and dry weather (2004, 2009, 2015), while others (2004 and 2005) were prolonged into the fall in the absence of season-ending rain events. In 2004, which was Alaska’s largest wildfire season at 6.6 million acres, the trajectory was characterized by both rapid mid-season growth and extended activity into September. These different pathways to large fire seasons demonstrate the importance of intraseasonal weather variability and the timing of dynamical features. As another example, although not large in total acres burned, the 2016 wildland fire season in Alaska was more than 6 months long, with incidents requiring response from mid-April through late October (AICC, 2016).
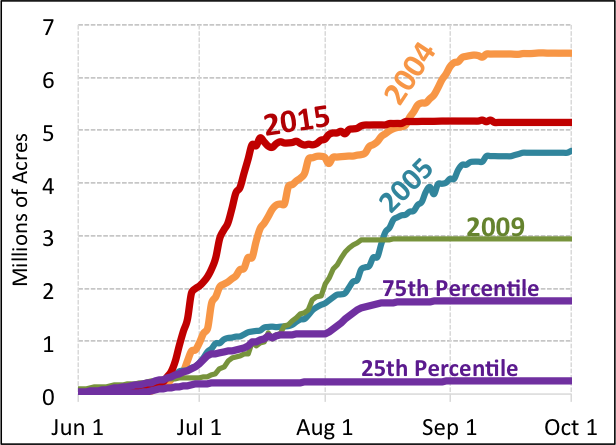
The snow-free season is increasing by approximately 5 days per decade in Alaska (Liston and Hiemstra, 2011), and the state’s fire management agencies advanced the statutory date of fire season from May 1 to April 1 in 2006 to better prepare for early events. In addition to tracking long-term trends and the impacts of a shifting climate, managers in Alaska and Canada must prepare for day-to-day variability in threats to its dispersed population with very limited resources. The Canadian Forest Fire Weather Index (FWI) System is used on a daily basis to estimate the spatial and temporal distribution of wildfire potential from observed and forecasted weather conditions (Lawson and Armitage, 2008). Among its indices, the Buildup Index (BUI), based on cumulative scoring of daily temperature, relative humidity, and precipitation, represents seasonal variability in fuel availability and flammability (Fig. 4). A threshold of 80 has been identified as a critical indicator of fire growth potential throughout the fire-prone landscape (Ziel et al., 2015).
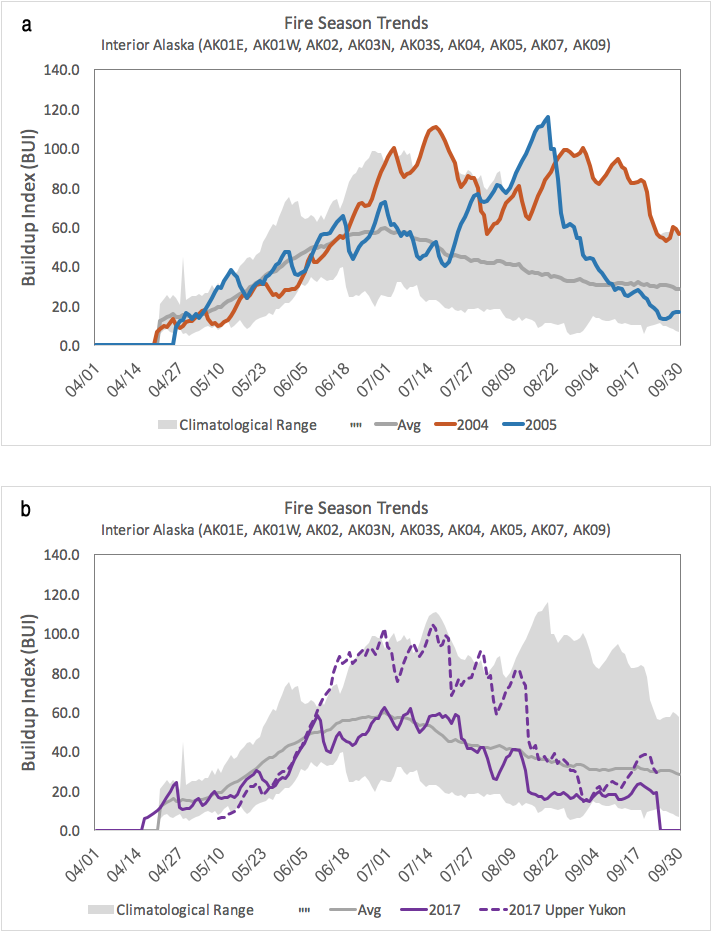
Figure 4a shows trends in BUI over selected seasons (2004 and 2005) in interior Alaska compared to the climatological range and average, derived from combined records from 116 surface observing locations from 1994-2017. Values for BUI in 2004 were elevated for a prolonged period in late June and July, and in both 2004 and 2005 reached extreme levels into August and September, consistent with the well above normal area burned late in those seasons (Figs. 1a and 3). In contrast, BUI values in 2015 (data not shown) were elevated in early to mid-season, coincident with the relatively brief period of extremely rapid fire growth, sparked by abundant lightning ignitions, seen in June and early July (Fig. 3).
In 2017, the typical acreage burned in Alaska (Fig. 1) was reflected by a fairly normal BUI trend across the boreal region that essentially paralleled the climatological average (Fig. 4b). However, it is noteworthy to reflect on how disproportionately the impact of a “normal” season can fall on specific areas in a landscape this large. For instance, while there were no significant peaks in the overall trend, local conditions in the Upper Yukon zone in northeast Alaska were significantly warmer and drier. Consistent with the Upper Yukon BUI trend (Fig. 4b), the season was extended and fairly severe in that large corner of the state, with periods of high fire danger (BUI ≥ 80) from mid-June into mid-August. More than 410,000 acres (63% of the 2017 Alaska total) were burned in the Upper Yukon area during this period (AICC, 2017).
Under a range of climate change scenarios, analyses using multiple approaches project significant increases (up to four-fold) in area burned in high latitude ecosystems by the end of the 21st century (French et al., 2015; Young et al., 2017; Yue et al., 2015, and references therein). In addition, lightning frequency is projected to increase by 12+/-5% per °C of warming in the contiguous U.S. (Romps et al., 2014), and may increase correspondingly in high latitudes. Because specific fire events depend on multiple interacting factors, the resulting changes in high latitude fire regimes will vary greatly over space and time, but all evidence indicates that northern ecosystems will become increasingly susceptible to burning.
References
Alaska Interagency Coordination Center (AICC), 2015: 2015 Fall Fire Review Statistics Summary. https://fire.ak.blm.gov/content/aicc/stats/Alaska%20Fire%20Numbers.pdf.
Alaska Interagency Coordination Center (AICC), 2016: 2016 Alaska Fire Season. https://fire.ak.blm.gov/content/aicc/Statistics%20Directory/Previous%20Years%20Fall%20Fire%20Review%20Handouts/2016%20Fire%20Data/2016%20Alaska%20Fire%20Season.pdf.
Alaska Interagency Coordination Center (AICC), 2017: Situation Report. https://fire.ak.blm.gov/content/aicc/sitreport/current.pdf.
Barrett, K., T. Loboda, A. D. McGuire, H. Genet, E. Hoy, and E. Kasischke, 2016: Static and dynamic controls on fire activity at moderate spatial and temporal scales in the Alaskan boreal forest. Ecosphere, 7(11), e01572, doi: 10.1002/ecs2.1572.
Bond-Lamberty, B., S. D. Peckham, D. E. Ahl, and S. T. Gower, 2007: Fire as the dominant driver of central Canadian boreal forest carbon balance. Nature, 450(7166), 89-92.
French, N. H. F., L. K. Jenkins, T. V. Loboda, M. Flannigan, R. Jandt, L. L. Bourgeau-Chavez, and M. Whitley, 2015: Fire in arctic tundra of Alaska: past fire activity, future fire potential, and significance for land management and ecology. International Journal of Wildland Fire, http://dx.doi.org/10.1071/WF14167.
Gillett, N. P., A. J. Weaver, F. W. Zwiers, and M. D. Flannigan, 2004: Detecting the effect of climate change on Canadian forest fires. Geophysical Research Letters, 31, L18211.
Johnstone, J. F., T. N. Hollingsworth, F. S. Chapin, and M. C. Mack, 2010: Changes in fire regime break the legacy lock on successional trajectories in Alaskan boreal forest. Global Change Biology, 16, 1281-1295, doi: 10.1111/j.1365-2486.2009.02051.x.
Jones, B., G. Grosse, C. D. Arp, E. A. Miller, L. Liu, D. J. Hayes, and C. F. Larsen, 2015: Recent Arctic tundra fire initiates widespread thermokarst development. Scientific Reports, 5. 15865, doi: 10.1038/srep15865.
Jorgenson, M. T., V. E. Romanovsky, J. Harden, Y. Shur, J. O’Donnell, E. A. G. Schuur, M. Kanevskiy, and S. Marchenko, 2010: Resilience and vulnerability of permafrost to climate change. Canadian Journal of Forest Research, 2010, 40, 7, 1219-1236. https://doi.org/10.1139/X10-060.
Kasischke, E. S., K. P. O’Neill, N. H. F. French, and L. L. Bourgeau-Chavez, 2000: Controls on patterns of biomass burning in Alaskan boreal forests, in Fire, Climate Change, and Carbon Cycling in the Boreal Forest, pp 173-196, Springer: New York.
Kasischke, E. S., and M. R. Turetsky, 2006: Recent changes in the fire regime across the North American boreal region-spatial and temporal patterns of burning across Canada and Alaska. Geophysical Research Letters, 33, L09703.
Lawson, B..D., and O. B. Armitage, 2008: Weather guide for the Canadian Forest Fire Danger Rating System. Nat. Resour. Can., Can. For. Serv., North. For. Cent., Edmonton, AB.
Liston, G. E. and C. A. Hiemstra, 2011: The changing cryosphere: Pan-Arctic snow trends (1979-2009). Journal of Climate, 24, 5691-5712.
Liu, H., J. T. Randerson, J. Lindfors, and F. S. Chapin, 2005: Changes in the surface energy budget after fire in boreal ecosystems of interior Alaska: An annual perspective. Journal of Geophysical Research, 110(D13), doi: 10.1029/2004JD005158.
Mann, D. H., T. S. Rupp, M. A. Olson, and P. A. Duffy, 2012: Is Alaska’s Boreal Forest Now Crossing a Major Ecological Threshold? Arctic, Antarctic, and Alpine Research, 44, 3, 319-331. https://doi.org/10.1657/1938-4246-44.3.319.
Northwest Territories Department of Environment and Natural Resources (NWT), 2015: 2014 NWT Fire Season Review Report. http://www.enr.gov.nt.ca/sites/enr/files/web_pdf_fmd_2014_fire_season_review_report_4_may_2015.pdf.
Partain, J. L., S. Alden, U. S. Bhatt, P. A. Bieniek, B. R. Brettschneider, R. T. Lader, P. Q. Olsson, T. S. Rupp, H. Strader, R. L. Thoman, J. E. Walsh, A. D. York, and R. H. Ziel, 2016: An assessment of the role of anthropogenic climate change in the Alaska fire season of 2015 [in “Explaining Extremes of 2015 from a Climate Perspective”]. Bulletin of the American Meteorological Society, 97 (12), S14-S18, doi: 10.1175/BAMS-D-16-0149.1.
Reid, C.E., M. Brauer, F. H. Johnston, M. Jerrett, J. R. Balmes, and C. T. Elliott, 2016: Critical review of health impacts of wildfire smoke exposure. Environmental Health Perspectives, 124, 1334-1343, http://dx.doi.org/10.1289/ehp.1409277.
Rogers, B. M., A. J. Soja, M. L. Goulden, and J. T. Randerson, 2015: Influence of tree species on continental differences in boreal fires and climate feedbacks. Nature Geoscience, 8(3), 228-234, doi: 10.1038/ngeo2352.
Romps, D. M., J. T. Seeley, D. Vollaro, and J. Molinari, 2014: Projected increase in lightning strikes in the United States due to global warming. Science, 346, 851-854.
Scharlemann, J. P., E. V. Tanner, R. Hiederer, and V. Kapos, 2014: Global soil carbon: understanding and managing the largest terrestrial carbon pool. Carbon Management, 5, 81-91.
Thomas, J. L., et al., 2017: Quantifying black carbon deposition over the Greenland ice sheet from forest fires in Canada. Geophysical Research Letters, 44, 7965-7974, doi: 10.1002/2017GL073701.
Turetsky, M., B. Benscoter, S. Page, G. Rein, G. R. van der Werf, and A. Watts, 2015. Global vulnerability of peatlands to fire and carbon loss. Nature Geoscience, 8(1), 11-14,. doi: 10.1038/NGEO2325.
Veraverbeke, S., B. M. Rogers, M. L. Goulden, R. R. Jandt, C. E. Miller, E. B. Wiggins, and J. T. Randerson, 2017: Lightning as a major driver of recent large fire years in North American boreal forests. Nature Climate Change, 7, 529-534, doi: 10.1038/nclimate3329.
Young, A. M., P. E. Higuera, P. A. Duffy, and F. S. Hu, 2017: Climatic thresholds shape northern high-latitude fire regimes and imply vulnerability to future climate change. Ecography, 40, 606-617, http://dx.doi.org/10.1111/ecog.02205.
Yue, X., L. J. Mickley, J. A. Logan, R. C. Hudman, M. V. Martin, and R. M. Yantosca, 2015: Impact of 2050 climate change on North American wildfire: consequences for ozone air quality. Atmospheric Chemistry and Physics, 15, 10033-10055, doi: 10.5194/acp-15-10033-2015.
Ziel, R. H., et al., 2015: Modeling fire growth potential by emphasizing significant growth events: characterizing climatology of fire growth days in Alaska’s boreal forest. 11th Symp. on Fire and Forest Meteorology, Minneapolis MN, Amer. Meteor. Soc., 1.2. [Available online at https://ams.confex.com/ams/11FIRE/webprogram/Paper272864.html.]
December 5, 2017