H. E. Epstein1, U. S. Bhatt2, M. K. Raynolds3, D. A. Walker3, B. C. Forbes4, M. Macias-Fauria5, M. Loranty6, G. Phoenix7, J. Bjerke8
1Department of Environmental Sciences, University of Virginia, Charlottesville, VA, USA
2Geophysical Institute, University of Alaska Fairbanks, Fairbanks, AK, USA
3Institute of Arctic Biology, University of Alaska Fairbanks, AK, USA
4Arctic Centre, University of Lapland, Rovaniemi, Finland
5School of Geography and the Environment, Oxford University, Oxford, UK
6Department of Geography, Colgate University, Hamilton, NY, USA
7Department of Animal and Plant Sciences, University of Sheffield, Sheffield, UK
8Norwegian Institute for Nature Research, Tromsø, Norway
Highlights
- Tundra greenness increased throughout the Arctic in 2015 (relative to 2014), following 3-4 years of continuous declines; an exception was that time-integrated (TI)-NDVI (an indicator of vegetation productivity) continued to decrease in North America.
- Long-term trends (1982-2015) show greening on the North Slope of Alaska, in the southern Canadian tundra, and in much of the central and eastern Siberian tundra, and browning in western Alaska (Yukon-Kuskokwim Delta), the higher-Arctic Canadian Archipelago, and western Siberian tundra.
Vegetation in the Arctic tundra has been responding dynamically over the course of the last several decades to environmental changes, many of which are anthropogenically-induced. These vegetation changes throughout the circumpolar Arctic are not spatially homogeneous, nor are they temporally consistent (e.g. Bhatt et al. 2013), suggesting that there are complex interactions among atmosphere, ground (soils and permafrost), vegetation, and herbivore components of the Arctic system. Changes in Arctic tundra vegetation may have a relatively small impact on the global carbon budget through photosynthetic uptake of CO2, compared to changes in other carbon cycling processes (Abbott et al. 2016). However, tundra vegetation can have important effects on permafrost, hydrological dynamics, soil carbon fluxes, and the surface energy balance (e.g. Blok et el. 2010, Myers-Smith and Hik 2013, Parker et al. 2015). Tundra vegetation dynamics also control the diversity of herbivores (birds and mammals) in the Arctic, with species richness being positively related to vegetation productivity (Barrio et al. 2016). To improve our understanding of these complex interactions and their impacts on the Arctic and global systems, we continue to evaluate the state of the circumpolar Arctic vegetation.
Using Earth-Observing Satellites (EOS) with daily return intervals, we have had the capacity to monitor Arctic tundra vegetation continuously since 1982. We report on data from the Global Inventory Modeling and Mapping Studies (GIMMS) version 3g dataset (GIMMS 2013) based largely on the Advanced Very High Resolution Radiometer (AVHRR) sensor onboard NOAA satellites (Pinzon and Tucker 2014). The GIMMS product is a bi-weekly, maximum-value composited dataset of the Normalized Difference Vegetation Index (NDVI); NDVI is highly correlated with aboveground vegetation (e.g. Raynolds et al. 2012), or tundra “greenness.” We use two metrics based on the NDVI: MaxNDVI and TI-NDVI. Max NDVI is the peak NDVI for the year (growing season), and is related to yearly maximum aboveground vegetation biomass. TI (time-integrated) NDVI is the sum of the bi-weekly NDVI values for the growing season, and is related to the total aboveground vegetation productivity.
Examining the overall trend in tundra greenness for the 34-year record, we find that both MaxNDVI and TI-NDVI have increased on the North Slope of Alaska, in the southern Canadian tundra, and in much of the central and eastern Siberian tundra, whereas tundra greenness has decreased (i.e. “Arctic browning”) in western Alaska (Yukon-Kuskokwim Delta), the higher-Arctic Canadian Archipelago, and western Siberian tundra (Fig. 7.1). Using the same NDVI dataset (albeit with a different vegetation map and a slightly shorter duration–1982-2012), Loranty et al. (2016) found that a much greater fraction of tundra areas overlying continuous permafrost exhibited long-term greening (42%) compared to browning (5%); in tundra areas overlying discontinuous permafrost the areal difference was not as great (27% greening and 10% browning). Across Arctic vegetation types (from 1982-2014), greening has been most extensive in forest – tall shrub tundra, moderately extensive in shrub tundra and sedge tundra, and minimal in low-lying shrub tundra. Interestingly, forest – tall shrub tundra also had the greatest fractional area of browning among the vegetation types, although the area of browning was <8% that of the area greening (Park et al. 2016). If we assess the 34-year trends with more temporal detail, both the North American and Eurasian Arctic have shown substantial increases in tundra greenness up to the early 2010s for MaxNDVI and the early 2000s for TI-NDVI. Since then, declines are visible in these tundra greenness indices (Fig. 7.2).
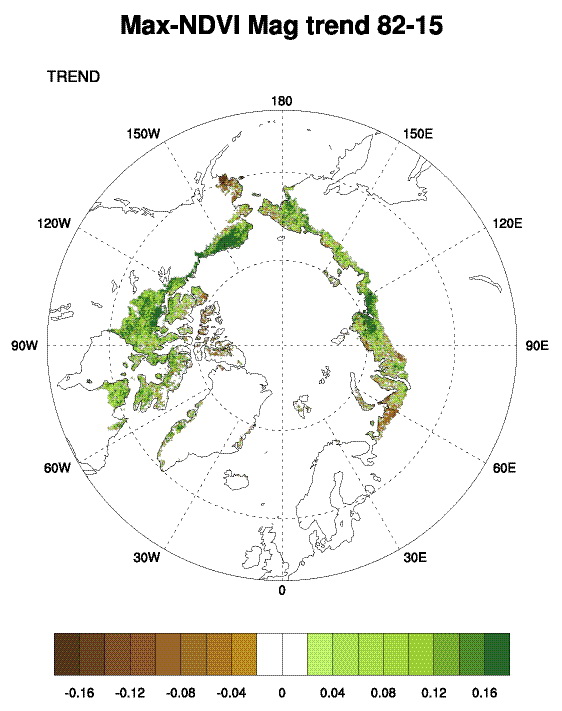
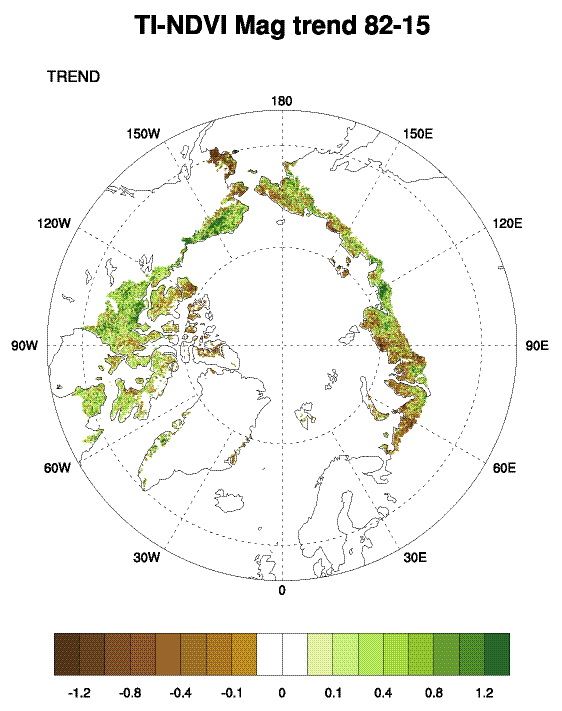
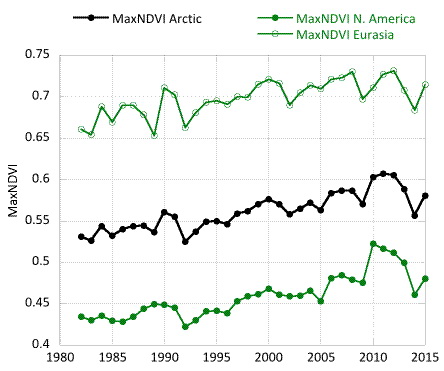
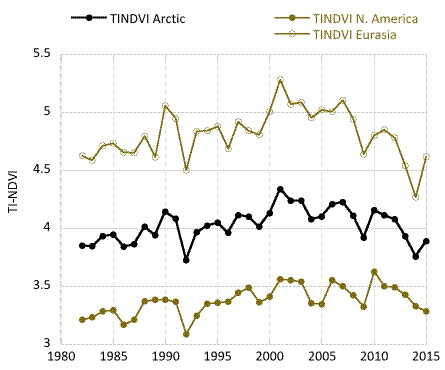
Following 3-4 years of successive declines (depending on the index and the continent), the NDVI for Arctic tundra exhibited an upturn during the summer of 2015, with the exception of TI-NDVI for North America, which continued to decrease (Fig. 7.2). Based on remotely-sensed Land Surface Temperatures (LST), from the same sensors as those providing the NDVI values, cumulative summer warmth (sum of mean monthly temperatures > 0° C) for the Arctic as a whole (and for the two continents separately) was greater in 2015 than in any other year of the satellite record (since 1982). Correspondingly, MaxNDVI increased by 4.3% and TI-NDVI increased by 3.6% for the circumpolar Arctic in 2015, relative to 2014 values. MaxNDVI values in 2015 were greater than the mean values for the record (1982-2015), ranking 8, 7, and 9 for the Arctic, North American Arctic, and Eurasian Arctic respectively over the 34-year record. TI-NDVI values were below the mean for the entire record, ranking 28, 28, and 29 for the Arctic, North American Arctic, and the Eurasian Arctic respectively.
While research on tundra browning is at present relatively sparse, there may be a variety of mechanisms leading to browning. Tundra browning in certain Arctic regions has been attributed to cooler summer temperatures (Bhatt et al. 2013) and to deeper winter snow packs and potentially longer snow cover duration (Bieniek et al. 2015). Again using the same NDVI dataset, Park et al. (2016) noted that since 2000 there has been a shortening of the growing season in the northern high latitudes, due to both a delayed start and an earlier end to the season. In a recent Commentary, Phoenix and Bjerke (2016) propose that tundra browning could be more “event-driven” than greening, caused by fire (Bret-Harte et al. 2013), extreme winter warming (Bokhorst et al. 2011), other anomalous weather events (e.g. frost damage), as well as outbreaks of insect and fungal pests (Graglia et al. 2001, Bjerke et al. 2014). Another potential cause of tundra browning could be increases in herbivore populations (Pederson et al. 2013, Hupp et al. 2015, Barrio et al. 2016).
In a recent remote-sensing analysis of global terrestrial ecosystems, Seddon et al. (2016) indicated that the Arctic tundra has been highly sensitive to climate variability over the past 14 years, the length of the satellite-based Moderate Resolution Imaging Spectroradiometer (MODIS) record. They also suggest that this sensitivity is largely correlated with temperature and cloudiness, environmental variables that are presently being altered (and likely will continue to be altered) by anthropogenic climate change. Further, Seddon et al. (2016) report much greater vegetation sensitivity to climatic variability in the low and mid-latitude tundra regions than in the High Arctic, in agreement with other remote sensing results (Epstein et al. 2012) and those of Myers-Smith et al. (2015), based on in situ growth measurements. Fully understanding the mechanisms behind tundra vegetation dynamics (and subsequent effects on other system properties) will require further cooperative efforts in scientific monitoring (field and remote sensing), experimentation, and simulation modeling.
References
Abbott, B. W., J. B. Jones, E. A. G. Schuur, F. S. Chapin III, W. B. Bowden, M. S. Bret-Harte, H. E. Epstein, M. D. Flanagan, T. K. Harms, T. N. Hollingsworth, M. C. Mack, A. D. McGuire, S. M. Natali, A.V. Rocha, S. E. Tank, M. R. Turetsky, J. E. Vonk, K. P. Wickland, G. R. Aiken, H. D. Alexander, R. M. W. Amon, B. W. Benscoter, Y. Bergeron, K. Bishop, O. Blarquez, B. Bond-Lamberty, A. L. Breen, I. Buffam, Y. Cai, C. Carcaillet, S. K. Casey, J. M. Chen, H. Y. H. Chen, T. R. Christensen, L. W. Cooper, J. H. C. Cornelissen, W. J. de Groot, T. H. DeLuca, E. Dorrepaal, N. Fletcher, J. C. Finlay, B. C. Forbes, N. H. F. French, S. Gauthier, M. P. Girardin, S. J. Goetz, J. G. Goldhammer, L. Gough, P. Grogan, L. Guo, P. E. Higeura, L. Hinzman, F. S. Hu, G. Hugelius, E. E. Jafarov, R. Jandt, J. F. Johnstone, I. Karlsson, E. S. Kasischke, G. Kattner, R. Kelly, F. Keuper, G. W. Kling, P. Kortelainen, J. Kouki, P. Kuhry, J. Laudon, I. Laurion, R. W. Macdonald, P. J. Mann, P. J. Martikainen, J. W. McClelland, U. Molau, S. F. Oberbauer, D. Olefeldt, D. Paré, M. -A. Parisien, S. Payette, C. Peng, O. S. Pokrovsky, E. B. Rastetter, P. A. Raymond, M. K. Raynolds, G. Rein, J. F. Reynolds, M. Robard, B. M. Rogers, C. Schädel, K. Schaefer, I. K. Schmidt, A. Shvidenko, J. Sky, R. G. M. Spencer, G. Starr, R. G. Striegl, R. Teisserenc, L. J. Tranvik, T. Virtanen, J. M. Welker, and S. Zimov, 2016: Biomass offsets little or none of permafrost carbon release from soils, streams, and wildfire: an expert assessment. Environ. Res. Lett., 11, 034014.
Barrio, I. C., C. G. Bueno, M. Gartzi, E. M. Soininen, K. S. Christie, J. D. M. Speed, V. T. Ravolainen, B. C. Forbes, G. Gauthier, T. Horstkotte, K. S. Hoset, T. T. Høye, I. S. Jónsdóttir, E. Lévesque, M. A. Mörsdorf, J. Olofsson, P. A. Wookey, and D. S. Hik, 2016: Biotic interactions mediate patterns of herbivore diversity in the Arctic. Glob. Ecol. Biogoegr., doi:10.1111/geb.12470.
Bhatt, U. S., D. A. Walker, M. K. Raynolds, P. A. Bieniek, H. E. Epstein, J. C. Comiso, J. E. Pinzon, C. J. Tucker, and I. V. Polyakov, 2013: Recent declines in warming and arctic vegetation greening trends over pan-Arctic tundra, Remote Sens. (Special NDVI3g Issue), 5, 4229-4254; doi:10.3390/rs5094229.
Bieniek, P. A., U. S. Bhatt, D. A. Walker, M. K. Raynolds, J. C. Comiso, H. E. Epstein, J. E. Pinzon, C. J. Tucker, R. L. Thoman, H. Tran, N. Mölders, M. Steele, J. Zhang, and W. Ermold, 2015: Climate drivers linked to changing seasonality of Alaska coastal tundra vegetation productivity. Earth Interactions, 19, 19.
Bjerke, J. W., S. R. Karlsen, K. A. Hogda, E. Malnes, J. U. Jepsen, S. Lovibond, D. Vikhamar-Schuler, and H. Tommervik, 2014: Record-low primary productivity and high plant damage in the Nordic Arctic Region in 2012 caused by multiple weather events and pest outbreaks. Environm. Res. Lett., 9, 084006.
Blok, D., M. Heijmans, G. Schaepman-Strub, A. Kononov, T. Maximov, and F. Berendse, 2010: Shrub expansion may reduce summer permafrost thaw in Siberian tundra. Glob. Change. Biol., 16, 1296-305.
Bokhorst, S., J. W. Bjerke, L. E. Street, T. V. Callaghan, and G. K. Phoenix, 2011: Impacts of multiple extreme winter warming events on sub-Arctic heathland: phenology, reproduction, growth, and CO2 flux responses. Glob. Change. Biol., 17, 2817-2830.
Bret-Harte, M. S., M. C. Mack, G. R. Shaver, D. C. Huebner, M. Johnston, C. A. Mojica, C. Pizano, and J. A. Reskind, 2013: The response of Arctic vegetation and soils following an unusually severe tundra fire. Phil. Trans. Royal Soc. B-Biol. Sci., 368, doi:10.1098/rstb.2012.0490.
Epstein, H. E., M. K. Raynolds, D. A. Walker, U. S. Bhatt, C. J. Tucker, and J. E. Pinzon, 2012: Dynamics of aboveground phytomass of the circumpolar arctic tundra over the past three decades. Environm. Res. Lett. 7, 015506.
Global Inventory Modeling and Mapping Studies (GIMMS), 2013: Available online: http://gcmd.nasa.gov/records/GCMD_GLCF_GIMMS.html.
Graglia, E., R. Julkunen-Titto, G. R. Shaver, I. K. Schmidt, S. Jonasson, and A. Michelsen, 2001: Environmental controls and intersite variations of phenolics in Betula nana in tundra ecosystems. New Phytologist, doi:10.1046/j.1469-8137.2001.00149.x.
Hupp, J. W., D. H. Ward, M. E. Whalen, and J. M Pearce, 2015: Changing arctic ecosystems – What is causing the rapid increase of Snow Geese in northern Alaska? U.S. Geological Survey Fact Sheet 2015-3062, http://dx.doi.org/10.3133/fs20153062.
Loranty, M. M., W. Liberman-Cribbin, L. T. Berner, S. M. Natali, S. J. Goetz, H. D. Alexander, and A. L. Kholodov, 2016: Spatial variation in vegetation productivity trends, fire disturbance, and soil carbon across arctic-boreal permafrost ecosystems. Environm. Res. Lett., 11, 095008.
Myers-Smith, I. H., S. C. Elmendorf, P. S. A. Beck, M. Wilmking, M. Hallinger, D. Blok, K. D. Tape, S. A. Rayback, M. Macias-Fauria, B. C. Forbes, J. D. M. Speed, N. Boulangier-Lapointe, C. Rixen, E. Lévesque, N. Martin Schmidt, C. Baittinger, A. J. Trant, L. Hermanutz, L. Siegwart Collier, M. A. Dawes, T. C. Lantz, S. Weijers, R. Haalfdan Jørgensen, A. Buchwal, A. Buras, A. T. Naito, V. Ravolainen, G. Schaepman-Strub, J. A. Wheeler, S. Wipf, K. C. Guay, D. S. Hik, and M. Vellend, 2015: Climate sensitivity of shrub growth across the tundra biome. Nature Climate Change, doi:10.1038/NCLIMATE2697.
Myers-Smith, I. H., and D. S. Hik, 2013: Shrub canopies influence soil temperatures but not nutrient dynamics: an experimental test of tundra snow-shrub interactions. Ecol. Evol. 3, 3683-700.
Park, T., S. Ganguly, H. Tømmervik, E. S. Euskirchen, K. -A. Høgda, S. R. Karlsen, V. Brovkin, R. R. Nemani, and R. B. Myneni, 2016: Changes in growing season duration and productivity of northern vegetation inferred from long-term remote sensing data. Environm. Res. Lett., 11, 084001.
Parker, T. C., J. -A. Subke, and P. A. Wookey, 2015: Rapid carbon turnover beneath shrub and tree vegetation is associated with low soil carbon stocks at a sub-arctic treeline. Glob. Change Biol., 21, 2070-2081.
Pedersen, Å. Ø., J. D. M. Speed, and I. M. Tombre. 2013: Prevalence of pink-footed goose grubbing in the arctic tundra increases with population expansion. Polar Biol., 36, 1569-1575.
Phoenix, G. K., and J. W. Bjerke, 2016: Arctic browning: extreme events and trends reversing arctic greening. Glob. Change. Biol., doi:10.1111/gcb.13261.
Pinzon, J., and C. Tucker, 2014: A non-stationary 1981-2014 AVHRR NDVI3g time series. Remote Sens., 6, 6929-6960, doi:10.3390/rs6086929.
Raynolds M. K., D. A. Walker, H. E. Epstein, J. E. Pinzon, and C. J. Tucker, 2012: A new estimate of tundra-biome phytomass from trans-Arctic field data and AVHRR NDVI. Remote Sens. Lett., 3, 403-411.
Seddon, A. W. R., M. Macias-Fauria, P. R. Long, D. Benz, and K. J. Willis, 2016: Sensitivity of global terrestrial ecosystems to climate variability. Nature 531, doi:10.1038/nature16986.
November 15, 2016